Studying Mobile Phases for HILIC UV/MS Analysis of Oligonucleotide Therapeutics including an siRNA Duplex, a Lipid Conjugated ASO, and a CRISPR sgRNA
Abstract
Hydrophilic interaction liquid chromatography (HILIC) has emerged as a promising alternative to traditional ion-pair reversed-phase (IP-RP) methods for oligonucleotide (ON) separations. This study explores the application of HILIC for the separation of ON diastereomers, duplexes, and single-stranded components, highlighting its compatibility with mass spectrometry (MS), thus eliminating the requirement of ion-pairing agents. Key parameters, including ionic strength, temperature, and mobile phase composition, were systematically optimized using design of experiments (DoE) to improve sensitivity. A sequenced injection procedure is also proposed to eliminate breakthrough effects and peak splitting.
Benefits
- HILIC provides an ion-pairing agent free chromatography method that can be easily applied with ammonium based mobile phases of differing concentration
- Although ionization efficiency is lower than HFIP based IP-RP LC-MS, but reliable LC-MS methods can be applied with mobile phase ionic strengths as low as 25 mM
- The GTxResolve™ Premier BEH™ Amide 300 Å 1.7 µm Column is batch tested and selected for its performance to separate both nucleic acids and proteins
- MaxPeak™ Premier Column hardware improves recovery and minimizes the need to passivate columns before use
Introduction
HILIC for Oligonucleotide Separations
Recently, hydrophilic interaction liquid chromatography (HILIC) has gained increasing interest for oligonucleotide (ON) separations.1,2,3 HILIC mode offers an attractive, sensitive, and robust alternative to the most commonly used ion-pairing reversed phase (IP-RP) methods. HILIC was found to be particularly promising for ON diastereomer separations. It has been shown that high ionic strength and low mobile phase temperature favor diastereomer selectivity.3 HILIC is also capable of separating duplex forms from their single-stranded components as it can be operated in either denaturing or non-denaturing (native) conditions depending on the mobile phase temperature.4 Another important advantage of HILIC is that it is favored for MS applications as it allows the use of ion-pair free mobile phases.5,6 HILIC has also been successfully applied for the bottom-up sequencing of sgRNAs in LC-MS and LC-MS/MS setups.7
Although robust methods can be developed in HILIC for ONs, the retention mechanism is not fully understood. Some authors mention that ionic interactions may be involved in the retention mechanism (note that it should be repulsive effect in this case), while others concluded that HILIC of ONs is mainly driven by hydrogen bonding interactions, either by adsorption or partitioning.1,3 It is thought that mobile phases with high ionic strength appear to stabilize the immobilized water layer on the surface of the polar (i.e. amide) stationary phase and minimize ionic interactions.3 However, some chromatographic models propose the opposite: when salts are added to aqueous mobile phases, water molecules will predominantly solvate salt ions (the highly ordered structure of water is disrupted), thus reducing the number of water molecules available to interact with a hydrophilic surface (expecting a reduction of the thickness of the immobilized water layer). It is also an interesting observation that HILIC columns provide good peak shape and similar selectivity when a protic co-solvent (i.e. methanol) is used instead of acetonitrile. This fact further complicates the understanding of the retention mechanism. Finally, it should be noted that the amount of water required to elute ONs from HILIC columns (20–60% water) is quite high, much higher than the amount required to elute glycans or glycoproteins (15–35% water).8
Experimental
LC Method Considerations
There is little mention of systematic method development for ON separations in HILIC. Very often the same or similar conditions are used (ammonium acetate, formate or bicarbonate in 10-50 mM and acetonitrile as co-solvent). The ionic strength of the mobile phase additive, temperature effects and pH have been studied, but very often separately (one factor at a time, OFAT) rather than in a combined design of experiments (DoE) or multidimensional retention model.1,3,9 Here, a systematic approach was used to compare the three ammonium salts. First, for screening purposes, a three-factor DoE was applied to study the effect of gradient steepness (gradient time, tG), temperature (T) and ionic strength (C)). The impact of tG and T was studied at two levels while the effect of C was studied at three levels. Then, the gradient program and temperature were simultaneously further optimized (using DryLab™ 4.4 software (Molnar Institute, Berlin, Germany)) to obtain a 10 minute separation that can be generally applied to different oligonucleotide ladder samples.
Breakthrough effect (or partial peak spliting) often occurs in HILIC due to an inherent mismatch between the sample diluent (biomolecules like proteins or oligonucleotides must be solubilized in aqueous media) and the mobile phase, which is mostly composed of aprotic solvent (acetonitrile).10 Practicing chromatographers often make a compromise to avoid breakthrough effects by diluting the sample in an acetonitrile-water mixture which can lead to sample precipitation and/or denaturation. Here we propose a more elegant solution: the addition of pre- and post injection plugs of weak solvent (acetonitrile) while the sample is injected in pure aqueous solvent plug (sequenced or ”bracketed” injection).
LC Analysis of Oligonucleotide Standards/Ladders
Sample preparation:
An oligo dT ladder was prepared by reconstituting the contents of a vial of MassPREP™ Oligonucleotide Standard (p/n: 186004135) in 100 µL water. A ssDNA ladder was prepared by reconstituting the contents of a vial of ssDNA Ladder 20–100 (p/n: 186009448) in 100 µL water. A lipid conjugated ASO oligonucleotide sample was prepared by reconstituting the contents of the Lipid Conjugated ASO LC-MS Standard (p/n: 186010747) in 20 µL of acetone and then diluted with 80 µL of water. A siRNA LC-MS standard (p/n: 186010598) was reconstituted in 100 µL water and used to study melting temperature in HILIC.
Mobile phase preparation:
HPLC grade water and acetonitrile (MeCN) were purchased from Fisher Scientific (Dublin, Ireland). Ammonium acetate, ammonium formate and ammonium bicarbonate were purchased from Sigma-Aldrich (Buchs, Switzerland). For the LC study, pre-mixed mobile phases were used to avoid precipitation and solubility problems. Mobile phase A was water/MeCN 30/70, while mobile phase B was water/MeCN 90/10. It was found that such a mobile phase composition could elute all oligonucleotide standards with adequate retention when running linear gradients of 0 - 100%B. To investigate the effect of additives in the mobile phase, three different ionic strengths (C1 = 10 mM, C2 = 25 mM, C3 = 50 mM) were considered for all three ammonium salts in both A and B.
For the final optimized LC-UV methods, 50 mM salts were added to both A and B mobile phases.
All other experimental conditions (LC-UV) are listed in the following table:
LC system: |
ACQUITY™ UPLC™ H-Class Bio System with Binary Solvent Manager [Equivalent to an ACQUITY Premier System with BSM FTN instrument configured with a High pH Kit] |
Detection: |
ACQUITY TUV Detector (Titanium Flow Cell, 5 mm, 1500 nL) |
Wavelength: |
260 nm |
Data acquisition: |
Empower™ Pro 3 Software Feature Release 3 |
Column: |
GTxResolve Premier BEH Amide Column, 300 Å, 1.7 µm, 2.1 x 50 mm Column (p/n: 186011249) |
Column temperature: |
50 °C for the optimized method (30 and 70 °C were set to model temperature effects) |
Sample temperature: |
6 °C |
Vials: |
QuanRecovery™ with MaxPeak™ HPS 12 x 32 mm Screw Neck Vials, 300 µL (p/n: 186009186) |
Injection volume: |
0.5–1 µL |
Flow rate: |
0.4 mL/min |
Mobile phase: |
A: 50 mM ammonium acetate/formate/bicarbonate in water/MeCN 30/70 B: 50 mM ammonium acetate/formate/bicarbonate in water/MeCN 90/10 |
Syringe draw rate: |
30 µL/min |
Needle placement: |
1.0 mm |
TUV sampling rate: |
20 Hz |
Filter time constant: |
none |
LC-MS Analysis of a Lipid Conjugated ASO and CRISPR sgRNA
Waters™ Lipid Conjugated ASO LC-MS Standard (p/n: 186010747) was first reconstituted in 20 µL of acetone then diluted with 80 µL of nuclease-free water. HPRT sgRNA was acquired from IDT and dissolved in nuclease-free water to a concentration of 50 pmol/µL.
LC system: |
BioAccord™ LC-MS System |
Detection: |
ACQUITY TUV Detector (Analytical Flow Cell) |
Wavelength: |
260 nm |
Data acquisition: |
Empower Pro 3 Software Feature Release 3 |
Column: |
GTxResolve Premier BEH Amide Column, 300 Å, 1.7 µm, 2.1 x 50 mm Column (p/n: 186011249) |
Column temperature: |
75 °C |
Sample temperature: |
6 °C |
Vials: |
QuanRecovery with MaxPeak HPS 12 x 32 mm Screw Neck Vials, 300 µL (p/n: 186009186) |
Injection volume: |
1 µL |
Flow rate: |
0.4 mL/min |
Mobile phase: |
A: 100 mM ammonium acetate in 18.2 MΩ water / 1% acetontrile (as prepared from IonHance Ammonium Acetate Concentrate p/n: 186009705 in a 1L Certified LDPE Reservoir p/n: 186009110) |
(sgRNA): |
B: LC-MS Grade Acetonitrile in a 1L Certified Glass Reservoir (p/n: 186007089) |
Mobile phase: |
A: 25 mM ammonium acetate in 18.2 MΩ water / 0.25% acetonitrile (as prepared from IonHance™ Ammonium Acetate Concentrate p/n: 186009705 in a 1L Certified LDPE Reservoir p/n: 186009110) |
(Lipid ASO): |
B: LC-MS Grade Acetonitrile in a 1L Certified Glass Reservoir (p/n: 186007089) |
Syringe draw rate: |
30 µL/min |
Needle placement: |
2.0 mm |
TUV sampling rate: |
20 Hz |
Filter time constant: |
none |
MS mode: |
Full Scan |
Mass range: |
High (400 to 5000 m/z) |
Scan rate: |
5 Hz |
Cone: |
40V |
Desolvation temperature: |
550 °C |
Gradient program for the LC-MS experiments
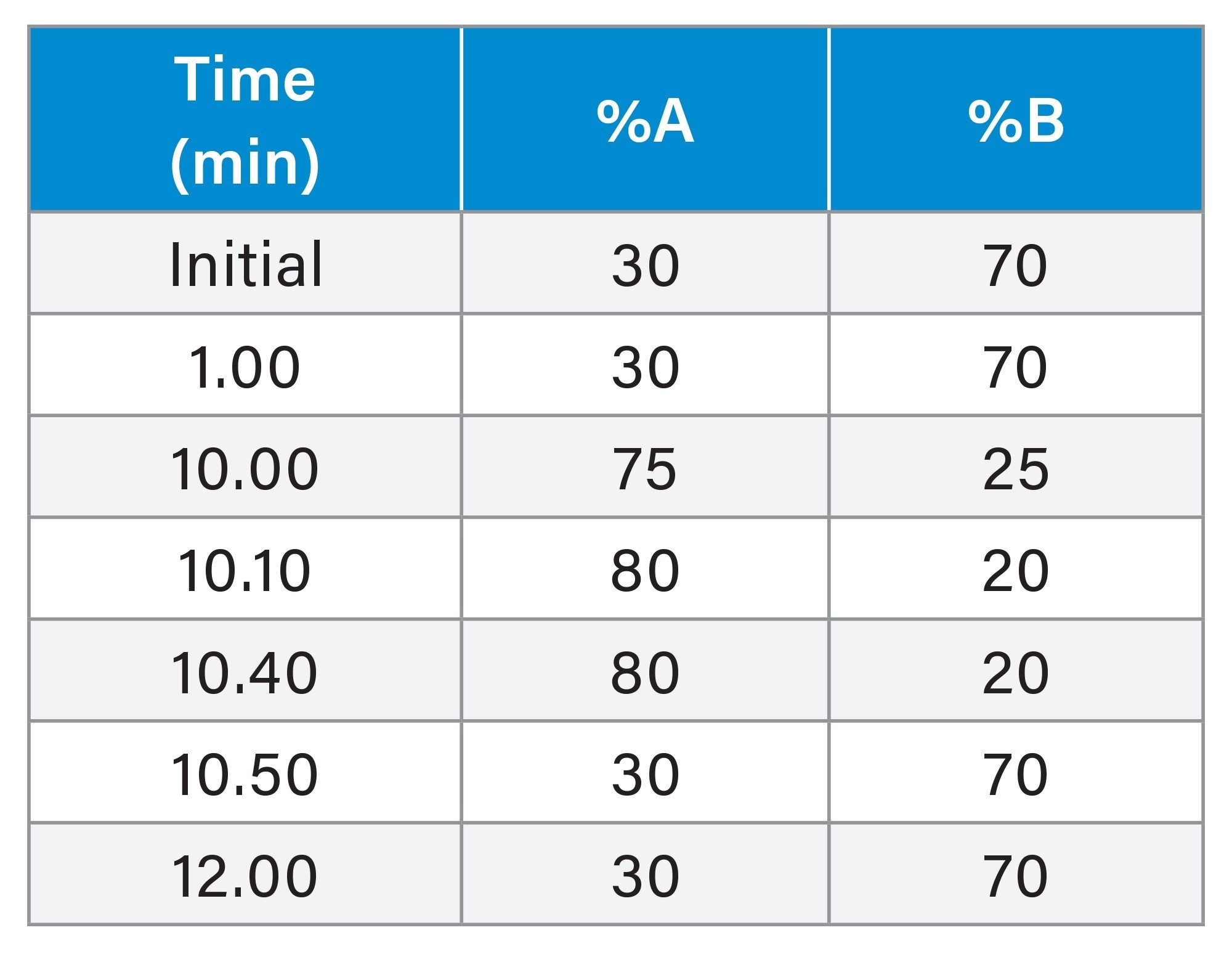
Results and Discussion
Comparison of Three Ammonium Salts as Mobile Phase Additives
The effect of ionic strength was found to be important when C ≤ 40 mM. When C ≤ 40 mM, retention decreases with decreasing ionic strength. If the additive concentration is too low (i.e. C = 10–20 mM), then breakthrough and/or peak splitting may be significant even if the retention of the first eluted peaks are set to be apparently high (i.e. the apparent retention factor is kapp ~ 5). Splitting and breakthrough become more severe at elevated temperatures.
In general, temperature was found to be a very important variable in tuning the selectivity and even the elution order of different ON species, so temperature is recommended to be optimized for a given sample.
The same considerations as for IP-RP can be applied to the gradient program in HILIC.11 Due to the homologous behaviour of ONs (i.e. ladders), the retention time of oligonucleotides will increase logarithmically rather than proportionally when running linear mobile phase gradients, so a logarithmic or concave gradient will inherently provide the highest possible selectivity. Figure 1 shows the chromatograms obtained with the three salts (additives) under optimized conditions - maintaining the same gradient steepness.
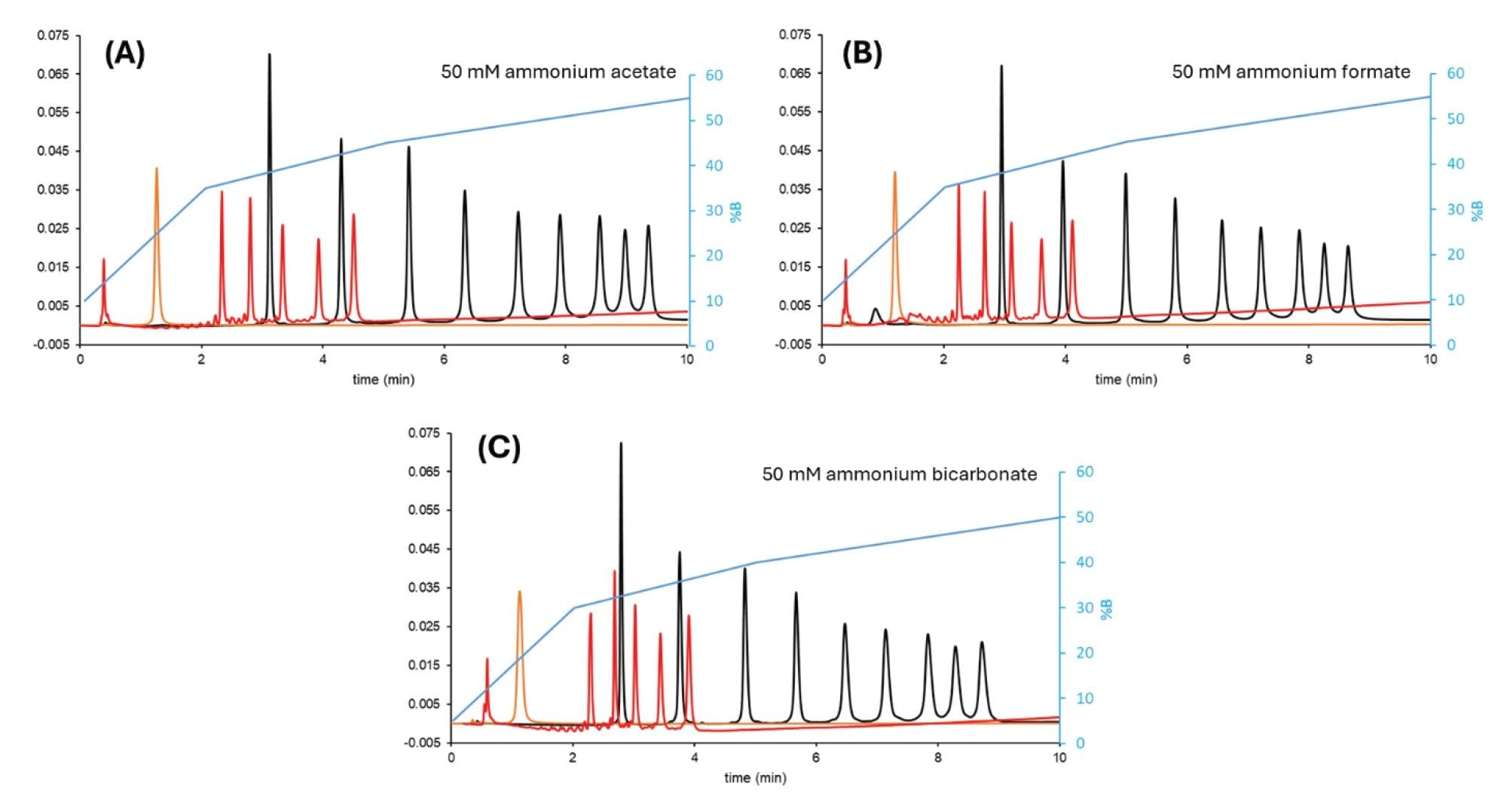
From a chromatographic separation point of view, ammonium acetate and formate are equivalent. The same gradient program can be used with both salts, resulting in almost identical retention and selectivity. However, ammonium bicarbonate results in lower retention than acetate or formate salts, so the gradient program must be adjusted (shifted by -5%B) to achieve a similar retention. Note that the selectivity is slightly different for bicarbonate. With bicarbonate, some early eluting peaks are more sensitive to peak splitting and breakthrough (especially at elevated temperatures) which is a disadvantage compared to the other two salts. However, this effect can be eliminated by using a bracketed injection (see in the next section).
Bracketed Injection to Avoid Breaktrough
Sample injection in HILIC can cause problems due to the higher eluent strength of the injection solvent compared to the mobile phase (solvent mismatch between the mobile phase composition and the injection solvent), as the sample is not sufficiently focused at the column inlet.10 Fine adjustment of the injection diluent could minimize this mismatch (i.e. increasing the percentage of acetonitrile in the sample diluent). However, this adjustment is not always possible due to the nature of the sample, which may cause denaturation, induction of post-translational modifications or even insolubility (precipitation). This solvent mismatch usually results in undesirable effects on the chromatographic separation, ranging from slight broadening to severe peak deformation or even splitting and analyte breakthrough. Breakthrough is a phenomenon where part of the sample migrates through the column with little interaction with the stationary phase and therefore elutes close to the column dead time, while the other part of the peak elutes at the normal retention time.
In our examples, the oligo dT ladder appeared to be the most sensitive to peak splitting. When the injection volume was set to 0.5 µL, the first compound (15 nt) eluted in a split peak. Above 1.5 µL injection volume, all five compounds (15, 20, 25, 30 and 35 nt) showed significant splitting and some of the solutes eluted at the column void.
A bracketed injection sequence can be helpful in eliminating breakthrough effects. In a bracketed injection, the sample is injected into the column head together with a pre and post plug of weak solvent (in this case MeCN). Using this bracketed injection, the sample injection volume could be increased up to 2 µL for the dT ladder without splitting. Figure 2 shows a comparison between normal and bracketed injections when 1 µL of sample is introduced into the flow path.
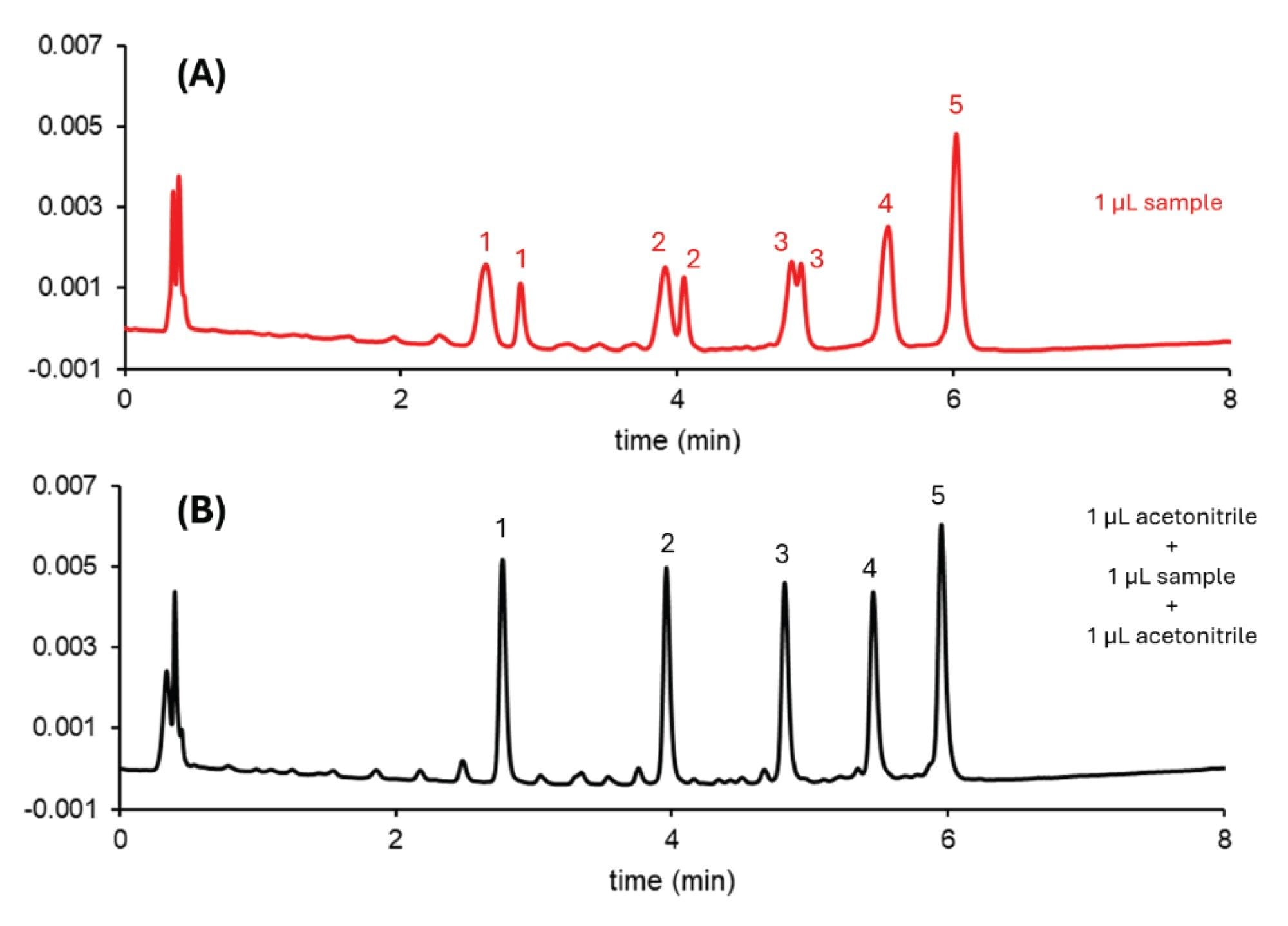
Temperature as a Key Parameter to Tune Separations
As well as tuning the selectivity and elution order of different species, temperature is also critical in setting non-denaturing or denaturing separation conditions in HILIC.
ON duplexes can be stabilized in non-denaturing conditions below the duplex melting temperature (Tm). Melting temperature is the property used to measure duplex stability; however, it is not fully understood how the chromatographic conditions affect the duplex stability.4 Pressure, temperature and co-solvents can significantly shift the apparent melting temperature.
A siRNA standard (mixture of annealed 25 mer and 27 mer RNA strands) was used to study the effect of temperature on duplex stability. Below 55 °C, a single peak was observed. At 60 °C, a very broad zone appeared on the chromatogram and its retention time decreased significantly compared to the sharp single peak (data not shown). And when the temperature was increased above 65 °C, two sharp peaks were observed on the chromatogram. These results suggest that this particular siRNA begins dissociating into its single strands at 60 °C. Figure 3 shows the temperature effects on duplex stability of a siRNA.
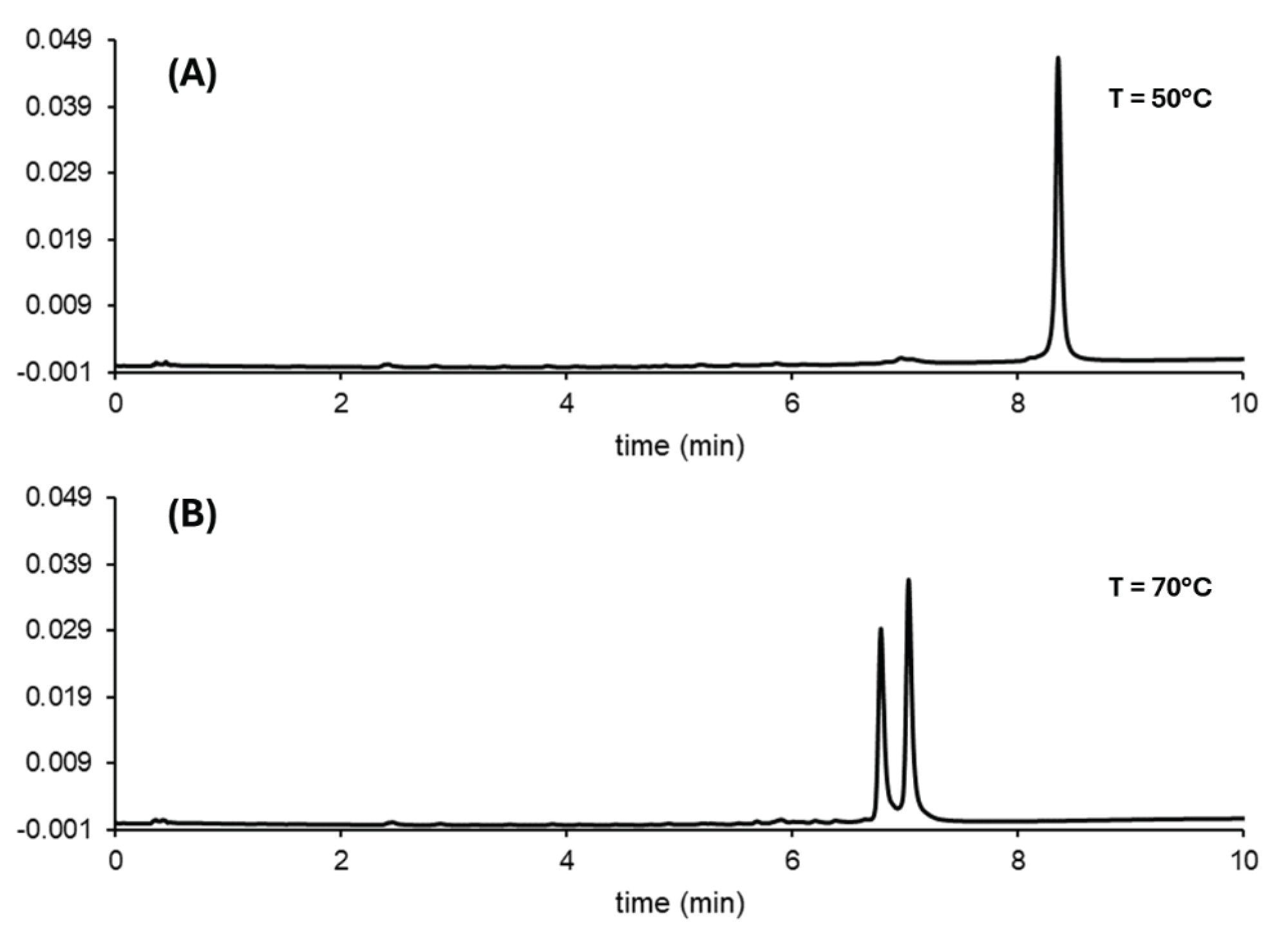
HILIC MS of a CRISPR sgRNA and Lipid Conjugated Antisense Oligonucleotide
With insights collected on the tunability of oligonucleotide HILIC methods, we next investigated the direct hyphenation of these separations with mass spectrometric detection. A BioAccord LC-MS System was applied to analyze a CRISPR sgRNA molecule as well as a lipid conjugated ASO, namely the Lipid Conjugated ASO LC-MS Standard. Two unique mobile phase composition were applied. In the case of the sgRNA, an aqeuous eluent modified with 100 mM ammonium acetate was applied. For the lipid conjugated ASO, a lower, 25 mM concentration of ammonium acetate was employed. Both cases produced effective levels of ion signal that could be directly interpretted with an accurate mass measurement derived from a monoisotopic peak or from MaxEnt deconvolution of the raw mass spectra.
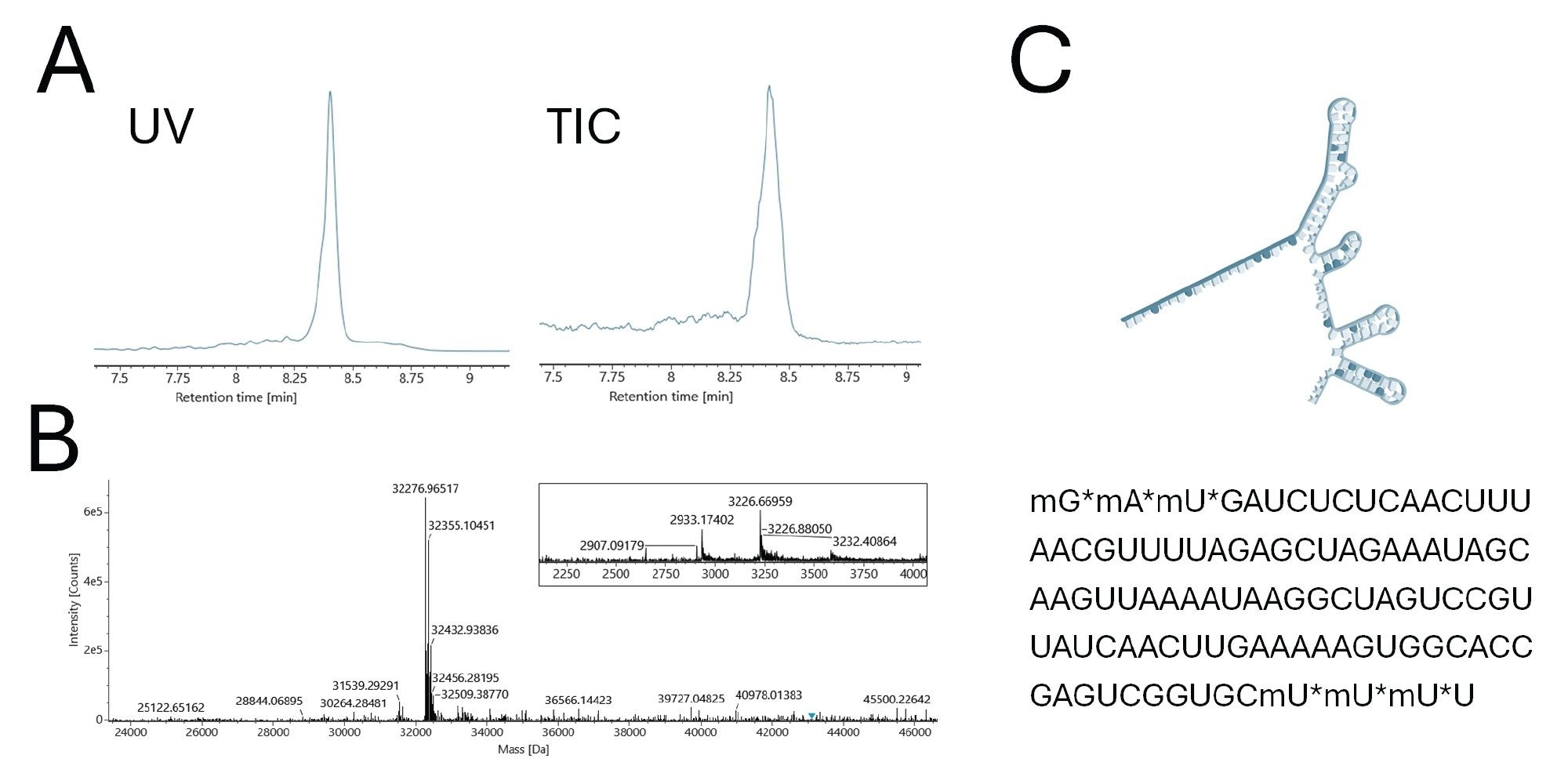
Figure 4 presents the LC-MS data collected for an CRISPR sgRNA synthesized with a human HPRT sequence complementarity. A peak was observed at 8.4 minute retention time. The MS information acquired for this peak is provided in panel B. The inset shows the summed, raw mass spectrum and a set of [M-11H+]11- and [M-10H+]10- charge state ions. The MaxEnt deconvoluted mass spectrum produced from this spectrum provided an sgRNA intact molecular weight of 32,276.97 Da, which is in close agreement to the sequence of this sgRNA inclusive of its 5’ and 3’ modified termini.
Results obtained for HILIC-MS analysis of the lipid conjugated ASO are included in Figure 5. In contrast to the sgRNA, the lipid ASO was not strongly retained on the HILIC column. This can be attributed to it being a signficantly smaller oligonucleotide that is conjugated to a C16 lipid. This lipid ASO contains a fully phosphorothioated backbone, along with several other modifications. A high temperature separation was found to reduce peak splitting from the sample’s diastereomers. With a final method using a 25 mM ammonium acetate eluent, two isobaric species were separated (Peaks 1 and 2). In addition, a sample impurity lighter in mass by 419 Da was resolved.
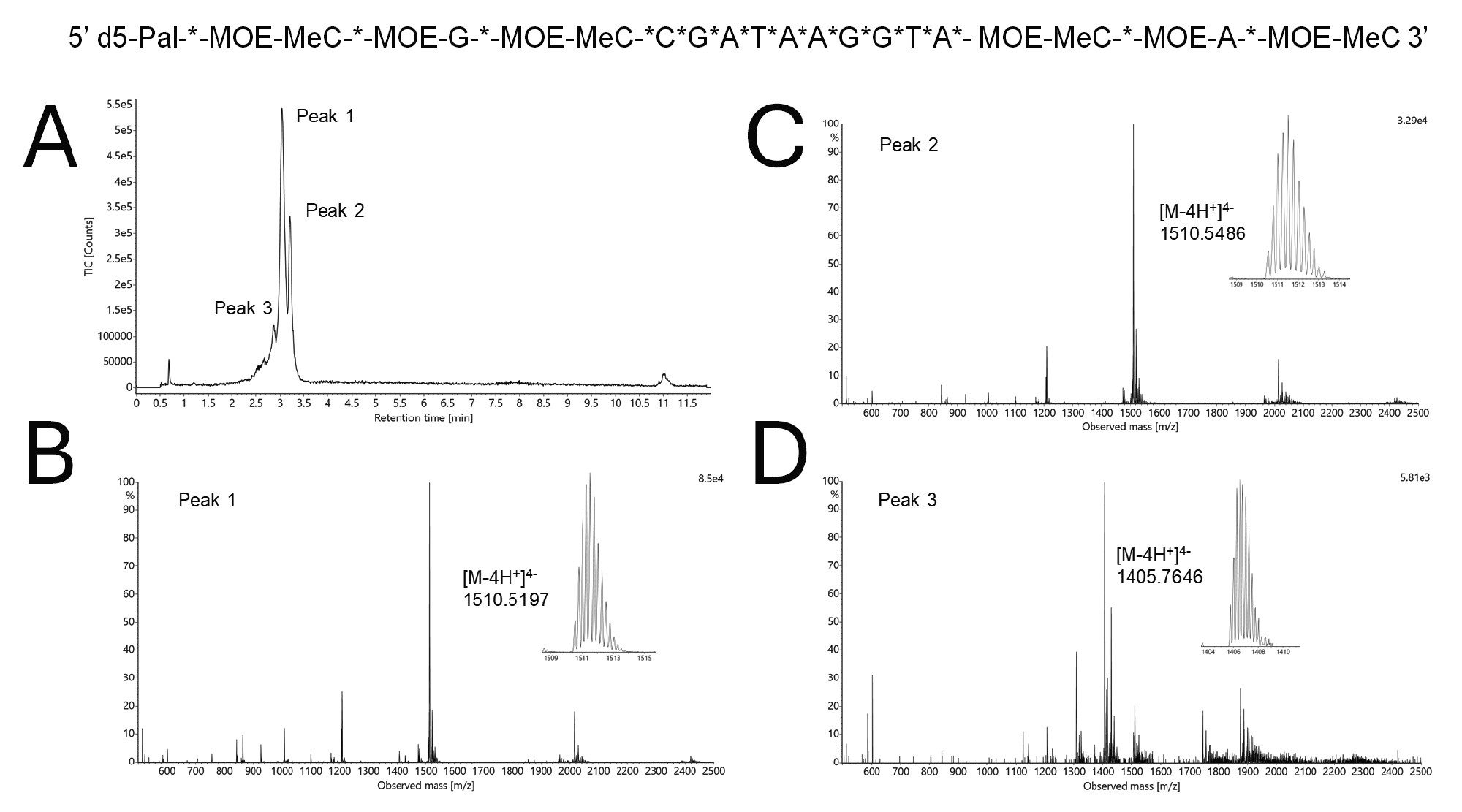
Conclusion
The results of this study demonstrate that HILIC offers several advantages for the separation and analysis of oligonucleotides and that HILIC methods are showing great potential for advancing analytical methods in the oligonucleotide therapeutics industry.
- Flexibility in Separation Conditions: HILIC can be operated in both denaturing and non-denaturing conditions, enabling the separation of duplex and single-stranded oligonucleotides, which is crucial for analyzing complex RNA structures and modifications.
- Compatibility with Mass Spectrometry: HILIC's ability to use ion-pair free mobile phases enhances compatibility with MS, improving sensitivity and accuracy in oligonucleotide sequencing and profiling, such as in bottom-up sequencing of sgRNAs.
- Efficient Method Development and Addressing Breakthrough Effects: The use of design of experiments (DoE) allowed for systematic optimization of critical parameters such as temperature, ionic strength, and gradient program, resulting in robust, reproducible separations. The bracketed injection method effectively mitigated sample breakthrough and peak splitting, allowing for higher injection volumes and improved chromatographic performance without compromising sample integrity.
HILIC represents a powerful and versatile tool for the separation and analysis of oligonucleotides, addressing key challenges faced by the oligonucleotide therapeutics industry. As the demand for more precise and sensitive analytical methods grows, especially with the rise of complex therapeutic modalities like antisense oligonucleotides (ASOs) and RNA-based therapies, HILIC offers a robust platform that ensures high resolution, MS compatibility, and flexibility in method development. Its application in quality control, sequencing, and impurity profiling will play a crucial role in accelerating the development of next-generation oligonucleotide therapeutics.
Waters, MassPREP, ACQUITY, UPLC, Empower, GTxResolve, BEH, QuanRecovery, MaxPeak, BioAccord and IonHance are trademarks of Waters Technologies Corporation. DryLab is a trademark of Molnar Institute. All other marks are the property of their respective owner.
References
- M. Gilar, B.M. Koshel , R.E. Birdsall, J. Chromatogr. A 1712 (2023) 464475. https://doi.org/10.1016/j.chroma.2023.464475
- A. Goyon, M.S. Blevins, J.G. Napolitano, D. Nguyen, M. Goel, B. Scott, J. Wang, S.G. Koenig, T. Chen, K. Zhang, J. Chromatogr. A 1708 (2023) 464327. https://doi.org/10.1016/j.chroma.2023.464327
- H. Lardeux, K. Stavenhagen, C. Paris, R. Dueholm, C. Kurek, L. De Maria, F. Gnerlich, T. Leek, W. Czechtizky, D. Guillarme, M. Jora, Anal. Chem. 96 (2024) 9994–10002. https://doi.org/10.1021/acs.analchem.4c01384
- M. Gilar, S. Redstone, A. Gomes, J. Chromatogr. A 1733 (2024) 465285. https://doi.org/10.1016/j.chroma.2024.465285
- P.A. Lobue, M. Jora, B. Addepalli, P.A. Limbach, J. Chromatogr. A 1595 (2019) 39–48. https://doi.org/10.1016/j.chroma.2019.02.016
- P. Anand, M. Koleto, D.R. Kandula, L. Xiong, R. MacNeill, Bioanalysis 14 (2022) 47–62. https://doi.org/10.4155/bio-2021-0216
- A. Goyon, B. Scott, K. Kurita, C.M. Crittenden, D. Shaw, A. Lin, P. Yehl, K. Zhang, Anal. Chem. 93 (2021) 14792−14801. https://doi.org/10.1021/acs.analchem.1c03533
- B. Bobaly, V. D’Atri, A. Goyon, O. Colas, A. Beck, S. Fekete, D. Guillarme, J. Chromatogr. B 1060 (2017) 325–335. http://dx.doi.org/10.1016/j.jchromb.2017.06.036
- H. Lardeux, V. D’Atri, D. Guillarme, TrACs 176 (2024) 117758. https://doi.org/10.1016/j.trac.2024.117758
- R. Perez-Robles, S. Fekete, Róbert Kormány, N. Navas, D. Guillarme, J. Chromatogr. A 1713 (2024) 464498. https://doi.org/10.1016/j.chroma.2023.464498
- S. Fekete, M. Imiolek, M. Lauber, Platform Ion Pairing RPLC Method for Oligonucleotides Using High Throughput 20 mm Length Columns, Waters Application Note, 720008460, August 2024.
720008602, November 2024